2023 February 26
Capturing Cosmic Rays with a Digital Camera
22 February 2023
Cosmic Rays are high-energy subatomic particles which fly through the Universe at speeds approaching the speed of light. They were discovered in the early 1900s by Wolf, Pacini, Hess, and Millikan, who carried out extensive research on ionizing radiation using shielded electrometers, which measure electric charge on a metal plate or a ball. They showed that ionizing radiation was present deep under ground and under water, that its levels were much higher at higher altitudes, and that the levels varied at different latitudes on the Earth’s surface. The results suggested that this radiation originated in outer space, and was composed of charged particles which are influenced by the Earth’s magnetic field. The extreme energy of this radiation was demonstrated by the fact that it penetrated without significant attenuation to electrometers enclosed in thick metal containers.
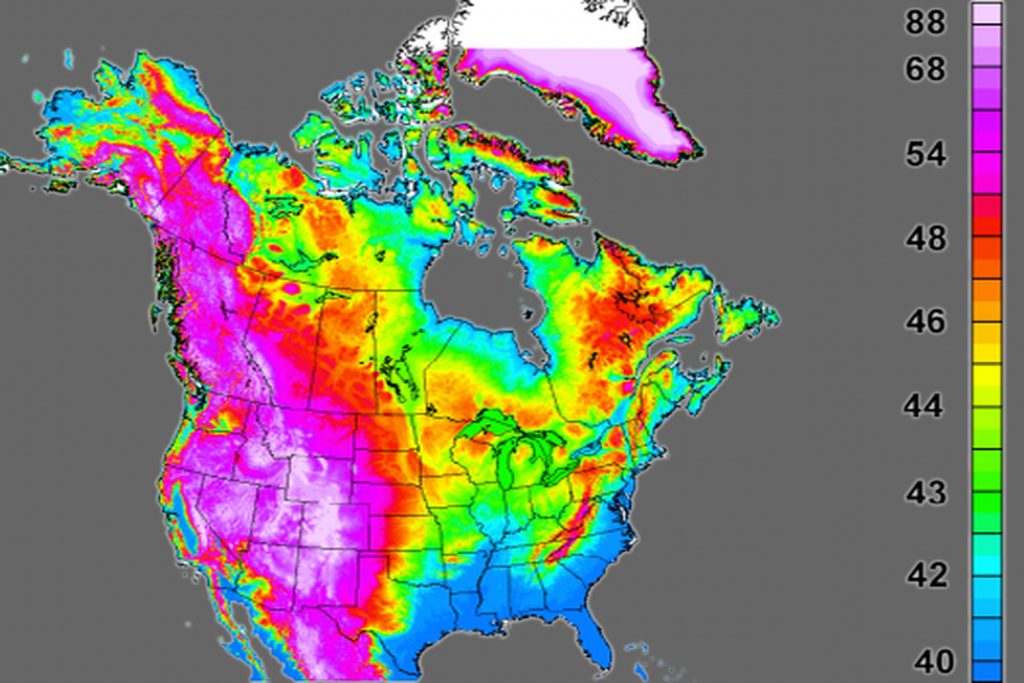
With the development in subsequent decades of high altitude air travel and space travel, research on cosmic rays and their effects on the human body and delicate electronic circuits became ever more important. By comparing ground-based and space-based research, it was confirmed that cosmic rays are not electromagnetic radiation, but subatomic particles which can be divided into two types: primary and secondary.
Primary cosmic rays are predominantly positively charged subatomic particles arriving from outer space at relativistic velocities. 88% are protons, or nuclei of Hydrogen. 10% are nuclei of Helium composed of two protons and two neutrons, also known as alpha particles. 1% are nuclei of heavier, more complex elements in the periodic table, present in approximately the same relative abundance as in the solar system. And only 1% are negatively charged isolated electrons. It is not well understood why negatively charged electrons might be less efficiently accelerated than positively charged atomic nuclei. One likely explanation is that electrons, having much lower mass and momentum, reach Earth in lower numbers because they are more easily captured around the magnetic field lines surrounding the source, and permeating interstellar space, the Solar System, and the Earth’s immediate environment. In the process of spiraling around magnetic field lines, the electrons generate synchrotron radiation which is readily detectable in many sources.
Recent satellite experiments reveal that a minute fraction of primary cosmic rays is composed of positrons and antiprotons arriving with no evidence of directionality. Investigation is ongoing in their origin and in the search for more complex antimatter particles.
In particle physics, energy is measured in electronvolts (eV). One eV is the energy change on a single electron as it moves across the electrical potential difference of 1 Volt, and is equal to approximately 1.6 x 10-19 joules (J). On the average, a primary cosmic ray particle carries the energy of 4.8 x 10-11 J, which corresponds to 3 x 108 eV, or to the velocity of 2/3 the speed of light. However, few particles move at nearly the speed of light, and carry the energy of 3 x 1020 eV, which is – incredibly – sufficient to light a 100W light bulb for 1 second. This is also equivalent to the kinetic energy of a baseball thrown at 100 miles per hour. By comparison, the highest energy particles produced by the upgraded Large Hadron Collider are in the range of 13 x 1012 eV, some 23 million times weaker.
The rate at which cosmic rays reach Earth is inversely proportional to their energy levels. While the least energetic ones rain upon the upper atmosphere at approximately 10,000 particles per square meter per second, the Very High Energy Cosmic Rays are fortunately very rare, with the estimated incidence of only a single event per square kilometer of Earth’s surface per century.
Low energy cosmic rays are generated on the surfaces of stars, as ionized particles are accelerated by the shock waves and rapidly expanding electromagnetic fields associated with flares and coronal mass ejections. Our Sun is an extremely volatile source. During periods of high solar activity, local flux of charged particles may increase between 100 and 1 million fold, and last up to several days. Meanwhile, high energy cosmic rays originate in the outer space. These particles are accelerated by immensely powerful and twisting electromagnetic fields around entities like the pulsars and the magnetars, or by cataclysmic events such as stellar collisions, supernova explosions, and accretion disks and polar jets generated by supermassive black holes in active galactic nuclei. Since cosmic ray particles are charged, and deviated by strong Galactic, interplanetary, and planetary magnetic fields, the direction of the original source can not be directly determined. Identification of numerous potential sources comes from the study of electromagnetic radiation in the radio, optical, and high energy bands, which are essentially unaffected by magnetic fields.
Secondary Cosmic Rays are formed when the primary cosmic rays enter the Earth’s atmosphere, collide with atoms and molecules in the air, and tear them apart in a process called spallation. The resulting air shower is a diverse mixture of nuclei of lighter elements, neutrons, and high energy subatomic particles which, in turn, decay into other particles of matter and antimatter, and into gamma ray photons.
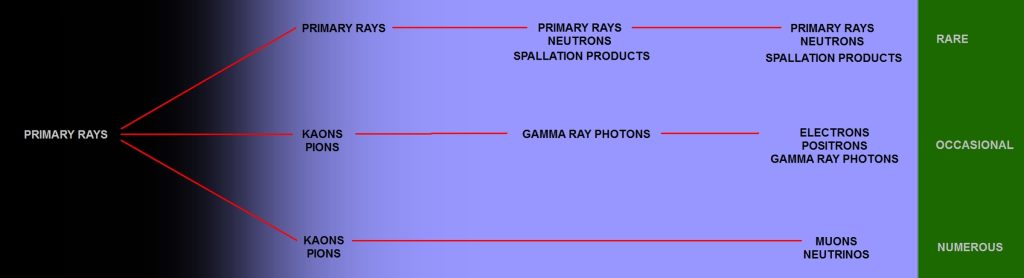
At the Earth’s surface, the most common secondary particles are Muons, negatively charged leptons, similar to electrons, but with 207 times greater rest mass. Other prevalent secondary and tertiary entities are electrons, positrons, neutrinos, and gamma ray photons. Only 3% of the cosmic rays on the surface are primary cosmic rays.
Muons are thought to be generated with the mean energy of 6 GeV (6 x 109 eV) at an altitude around 15,000 meters. They interact very little physically with ordinary matter but, since they carry a negative charge, they do lose energy by ionizing atoms along their path. The longer the path through a material, the greater the energy loss. At sea level, where they constitute about half the natural background radiation, the mean energy of muons is around 4 GeV. This allows them to penetrate deep under water, and over 700 meters under ground. To fully shield from most secondary cosmic rays would require a wall of iron 2 meters, or 6 feet thick.
Like other elementary particles, the muon can exist as ordinary matter, called negative muon, or antimatter, called antimuon or positive muon. Antimuons decay 100% of the time into positrons, antineutrinos, and neutrinos. However, negative muons of ordinary matter may follow one of two paths. Some decay into electrons, neutrinos, and antineutrinos, while others may rejoin matter by capture into very tight orbits around atomic nuclei where they combine with a proton to produce a neutron and a neutrino.
Muons are unstable elementary particles with a half-life of 2.2 µs (microseconds). This means that every 2.2 millionths of a second half of all the muons spontaneously decay into more stable particles. During that time light covers the distance of only 660 meters. Virtually no muons would survive long enough to traverse the 15,000 meter trip through the Earth’s atmosphere were it not for the relativistic effects of time dilation. Due to their velocity, which is near the speed of light, time on a muon passes about 40 times more slowly than in our frame of reference, and its half-life is proportionally longer. This allows it a sufficient life span to travel through the atmosphere.
Muons can be detected with cloud chambers, Geiger counters, and scintillator detectors, but can also be recorded with CCD and CMOS chips in ordinary digital cameras which are sensitive to charged particles. Muon flux at the surface of the Earth averages approximately 1 particle per square centimeter per minute. The surface area of the APS-C camera sensor (22.3 x 14.9 mm) is 3.32 cm2, which means that we can expect on average 3 muon strikes on the sensor during a 1 minute exposure.
The following method was used to obtain images listed below:
– Camera: Canon T3i (600D), lens removed and replaced with a light-tight body cap.
– Camera set to BULB for long exposures, with remote control shutter switch attached.
– Sensitivity set to ISO 1600. Higher sensitivities result in too much thermal noise. Less sensitivity results in less distinctive muon trails.
– Image quality in camera’s Menu set to maximum resolution 18M (5184×3456), JPG format.
– Long exposure noise reduction turned on. (Camera’s Menu / Custom Functions / C.FnII:Image / turn on Long exp. noise reduction.) This feature automaticaly takes an appropriate dark exposure to subtract hot pixels and amplifier glow from the original image. Some isolated defective or hot pixels may appear on long exposure photos at high sensitivity settings in spite of noise reduction. However, it is statistically extremely unlikely for several defective pixels to appear in a cluster or a row, adjacent to one another.
– On the Canon T3i (600D) camera, turning off Live View before taking a long exposure eliminates amplifier glow. This may not hold true with other camera models.
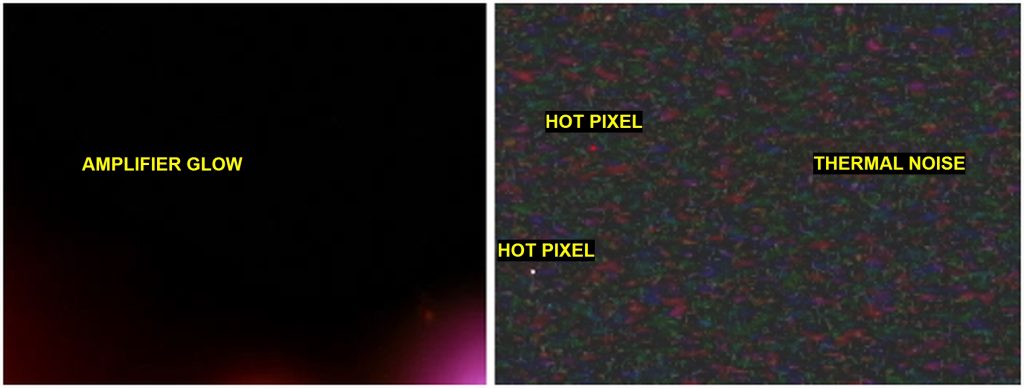
– Since most cosmic rays arrive at high angles to the horizon, the camera is placed on its back so that the CMOS sensor is horizontal, and maximally exposed to the cosmic ray flux.
– The length of exposures was 3 minutes. Longer exposures result in more thermal noise. Shorter exposures are less likely to capture cosmic rays. Following the original exposure, the camera will automatically take a dark frame of equal duration.
– Images, which appear entirely black at low magnification, are zoomed-in 5-10x (20-10% linear crop) with image processing software, slowly scanned, and examined for pixel clusters, tracks, and groups. These are further magnified to reveal muon trails similar to those shown below.
– Image processing software used here for magnification and cropping was XnView, but a number of other excellent programs are suitable.
Pixel clusters are produced when particles strike the sensor orthogonally; tracks when they strike at an angle; and groups when particles decay close to the sensor surface, before the resulting products had time to separate. There is no statistical confidence that single, isolated, illuminated pixels, or “dots”, are caused by muon collisions rather than residual defective or hot CMOS pixels. Isolated dots should be excluded from the muon count.
Several interesting cosmic ray studies can be performed with a digital camera.
The camera may be placed upright, with the sensor in the vertical position, which should, in theory, result in a higher incidence of tracks relative to clusters and groups, but fewer muon trails overall.
The camera may be covered with a thick metal pot, which should not result in the decrease in the average muon flux since muons readily pass through matter.
Daytime and night-time muon flux may be compared during periods of known high and low solar activity.
It is reasonable to assume that the total number of illuminated pixels in a cluster is proportional to the energy level of the incident muon. With data from a large number of muon trails it is possible to draw a histogram with the number of illuminated pixels on the X-axis, and the number of muon trails on the Y-axis. Such a histogram would roughly reflect the distribution of relative muon energy under the given experimental conditions.
If an opportunity presents to take measurements deep under ground, such as in mines or caves, muon flux and mean energy should be significantly lower than on the surface.
Conversely, taking measurements during a high altitude airplane flight should show more numerous and more energetic muon trails and, possibly, an occasional primary cosmic ray trail.
—
Some example images:
https://britastro.org/wp-content/uploads/2023/02/Clusters-01.jpg
https://britastro.org/wp-content/uploads/2023/02/Clusters-02.jpg
https://britastro.org/wp-content/uploads/2023/02/Clusters-04.jpg
https://britastro.org/wp-content/uploads/2023/02/Clusters-05.jpg
https://britastro.org/wp-content/uploads/2023/02/Clusters-06.jpg
https://britastro.org/wp-content/uploads/2023/02/Clusters-07.jpg
https://britastro.org/wp-content/uploads/2023/02/Clusters-08.jpg
https://britastro.org/wp-content/uploads/2023/02/Groups-01.jpg
https://britastro.org/wp-content/uploads/2023/02/Groups-02.jpg
https://britastro.org/wp-content/uploads/2023/02/Groups-03.jpg
https://britastro.org/wp-content/uploads/2023/02/Groups-04.jpg
https://britastro.org/wp-content/uploads/2023/02/Groups-05.jpg
https://britastro.org/wp-content/uploads/2023/02/Groups-06.jpg
https://britastro.org/wp-content/uploads/2023/02/Tracks-01.jpg
https://britastro.org/wp-content/uploads/2023/02/Tracks-02.jpg
https://britastro.org/wp-content/uploads/2023/02/Tracks-03.jpg
https://britastro.org/wp-content/uploads/2023/02/Tracks-04.jpg
https://britastro.org/wp-content/uploads/2023/02/Tracks-05.jpg
https://britastro.org/wp-content/uploads/2023/02/Tracks-06.jpg
https://britastro.org/wp-content/uploads/2023/02/Tracks-07.jpg
https://britastro.org/wp-content/uploads/2023/02/Tracks-08.jpg
https://britastro.org/wp-content/uploads/2023/02/Tracks-09.jpg
The British Astronomical Association supports amateur astronomers around the UK and the rest of the world. Find out more about the BAA or join us. |